Five decades ago, a scientist at a major oil company invented a device that would change the world. It would take a while to do so.
The scientist, M. Stanley Whittingham, created the first lithium-ion storage battery in 1972 while at Exxon. The oil company did not make much of the battery; it appeared in one trade show and then Exxon dropped it.
Academic researchers kept poking at the technology though, and so did commercially-minded inventors. By 1987, the battery found its first commercial home – Sony – and its first commercial application – its new camcorder, the Handycam.
Five decades on, the lithium-ion storage battery is ubiquitous. You are almost certainly reading this on a device that uses a small one; you may drive yourself in a vehicle that features a very large one. The three key creators of the device earned a Nobel Prize for its invention; in awarding them, Royal Swedish Academy of Sciences stated that John B. Goodenough, M. Stanley Whittingham, and Akira Yoshino “created a rechargeable world.” We can thank thousands of engineers for continuing to advance the power of these batteries, and perhaps hundreds of thousands of technicians and assemblers can be thanked for bringing this invention to ubiquity.
Today’s electrochemical battery is more than ubiquitous, however. It is now on its way to becoming a general-purpose technology for decarbonization. After decades of fundamental research, continuous innovation, and an ever-expanding application set, lithium-ion batteries in particular are now reshaping everything from the world’s billion-plus fleet of vehicles to the global power grid. Batteries are more than essential for tomorrow’s power and transport system; they are foundational. By separating energy production and consumption in time and space, batteries allow energy systems to manage power variability, time delivery for points of maximum value, and vastly increase the stock of renewable power generated and the flow of their generated electrons. Without widespread energy storage, economy-wide decarbonization will neither scale, nor endure.
Today’s batteries are just the start of a wave of innovation. In the next few years, we will see advances in chemistries, applications, and form factors that will dramatically expand on today’s use cases. These new devices will revolutionize transportation markets, the electric power system, and even what we wear in a world with deeply-integrated stored electric energy.
This letter examines the technology, processes, and markets that today’s batteries have created, and looks ahead to the technical and commercial innovations that emerging technologies will create.
Price, volume, quality
Lithium-ion batteries have followed a familiar trajectory for high-volume manufactured electronic goods: initially very high prices and small, often bespoke volumes eventually growing into mass volume and with it, much lower prices. On a volume-weighted basis, today’s battery used in an electric vehicle or stationary storage application costs a fifth of what it did a decade ago.
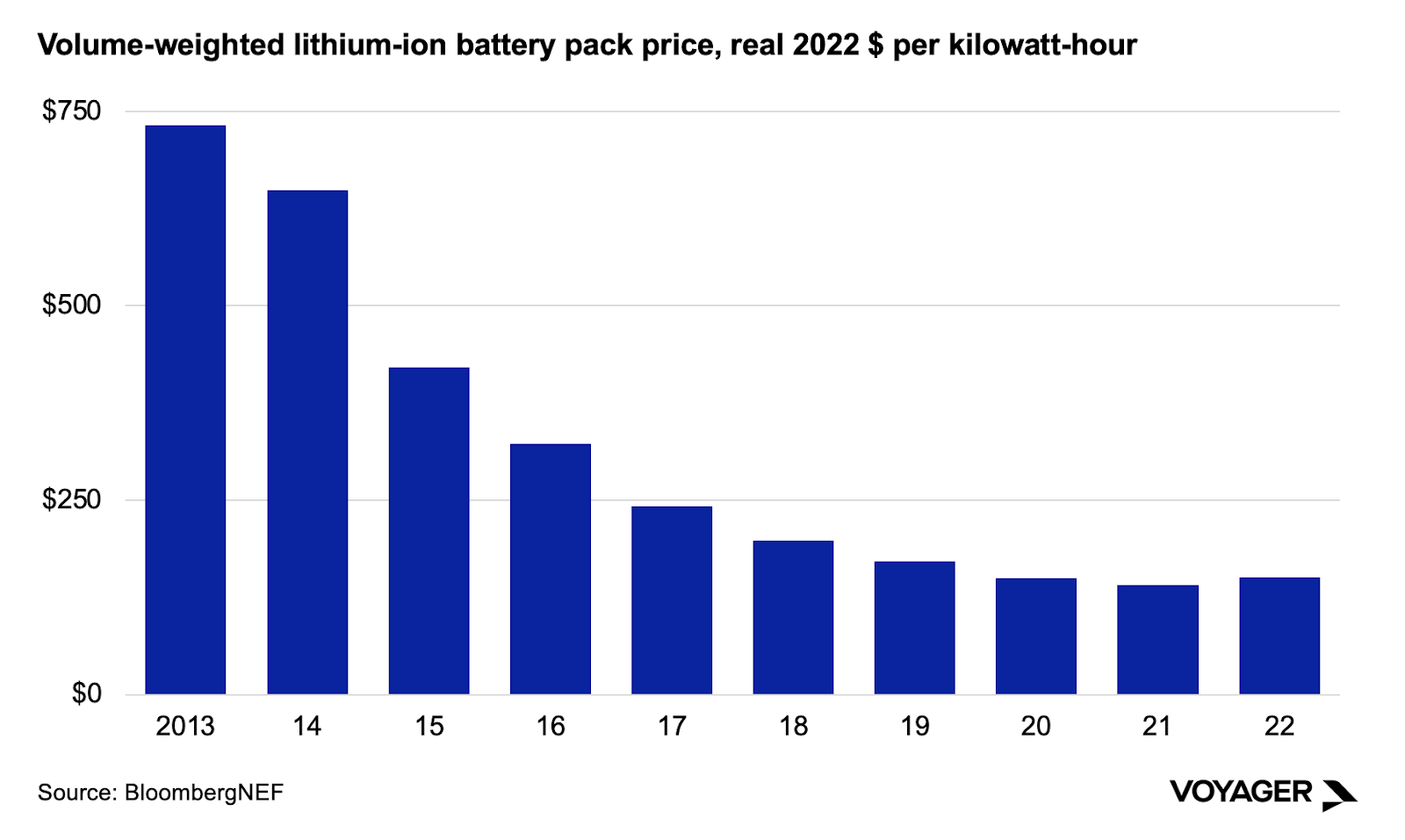
While stationary storage applications are an important battery use case today, vehicle applications overwhelmingly dominate total volume. Later in this letter, we will explore potential implications of this massive amount of stored energy on wheels. Last year, vehicle makers installed 550 gigawatt-hours of energy storage capacity in vehicles; the world’s stationary storage applications used about 35 times less capacity. The growth pattern is similar, but not the axes in the charts below.
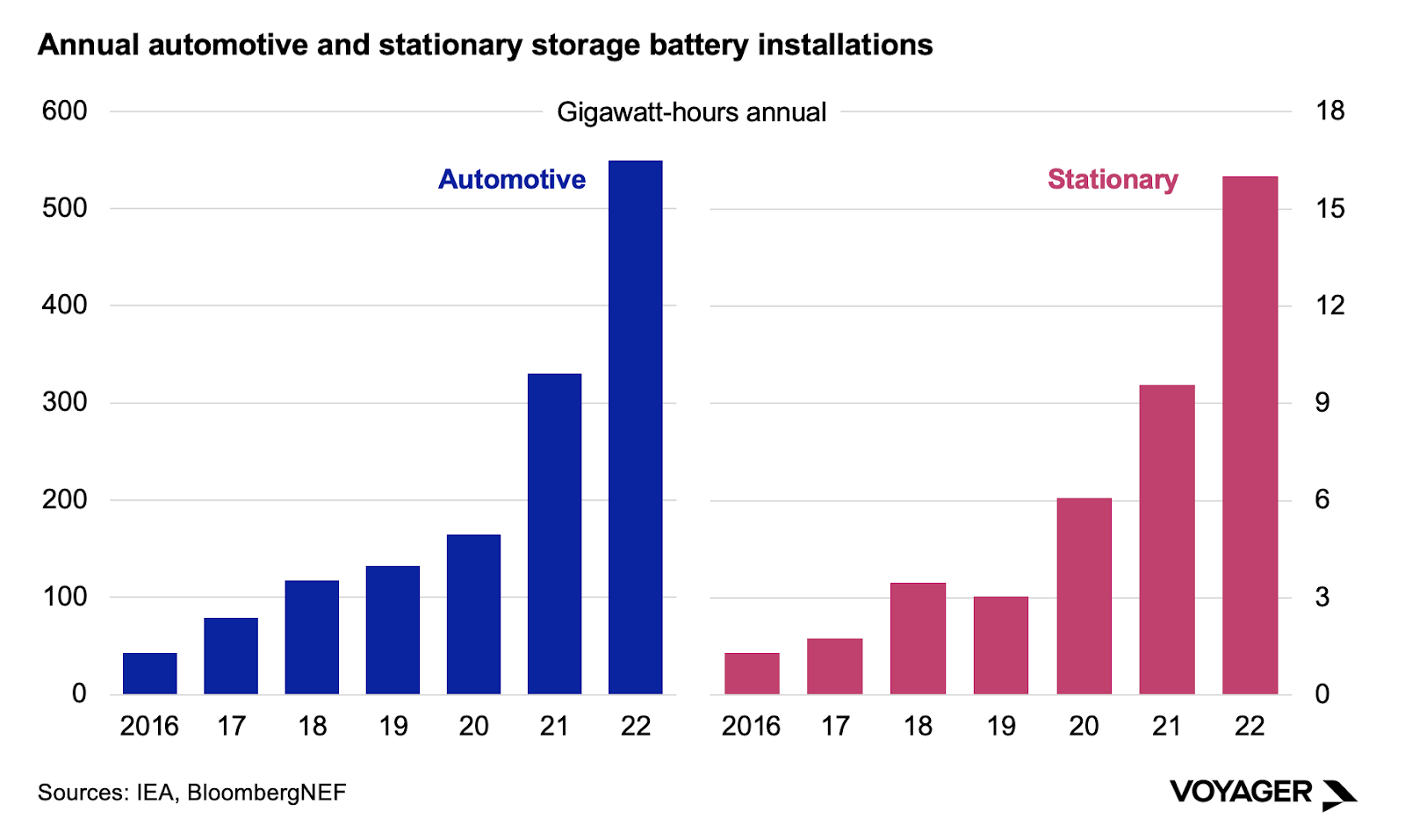
Today, there are multiple chemistries used in commercialized storage batteries. Each chemistry is suited to a purpose or set of purposes, but each has inherent tradeoffs as well. High energy density can also require scarce materials and also create greater safety risk; low costs can also mean lower energy density and less power portability. These tradeoffs are real, but market reception to them is fluid, in a useful way.
Companies and individuals owning and operating batteries will evaluate their needs and their priorities in choosing which batteries to deploy, and where. Some may prioritize the quick dispatch of stored power, which is useful for supporting the power grid during times of fluctuating frequency. Others may prioritize high energy density, in particular for vehicle applications. And others may prioritize low cost, either for cost-sensitive vehicle markets or for grid-tied applications where every dollar saved in capital expenditure translates to higher project IRRs. Price, storage duration, abundance of mineral inputs – and their geopolitical contexts, density, safety, and size all interact in the energy storage marketplace, and will continue to do so as chemistries improve in cost and efficiency.
Lithium-based chemistries
The dominant chemistry in today’s batteries is lithium-nickel-manganese-cobalt-oxide, or Li-ion NMC for short. This battery dominates most vehicle applications, be they in e-bikes or supercars. These batteries have the advantage of high energy density, but at the same time also use scarce metals that can be problematic to source - nickel and cobalt, in particular. NMC batteries have the potential for rapid ‘thermal runaway’, which can lead to dramatic, even deadly, fires when batteries are poorly-made or maintained. NMC’s high performance today brings with it a small but nonetheless evident high risk; manageable for the highest-quality, best-maintained applications but hard to envision as the dominant technology in a universe of hundreds of millions of small, more affordable vehicles.
The soaring price of lithium – as well as high prices for the nickel and cobalt that NMC batteries require – led vehicle makers to seek a viable commercial alternative chemistry using fewer scarce and expensive materials. The first version of that substitute is lithium iron phosphate (LFP).
As the name would suggest, LFP batteries eschew nickel and cobalt in favor of more abundant, and lower-cost, iron and phosphate. These metals result in a battery that has about half the energy density of NMC batteries, but also costs 30% less. LFP is proving well-suited to mass-market automotive applications, in particular in China, where LFP batteries were 62% of the market in 2022, doubling their share in less than a year. LFP batteries are also well-suited to stationary storage applications serving the power grid (and off-grid uses), where weight and space are not constrained.
Manufacturers are already looking beyond LFP for a chemistry that eclipse LFP as LFP is eclipsing NMC. Battery makers in China are now commercializing sodium-ion batteries, which have no lithium at all and instead use the sixth-most abundant element on earth. Perhaps more importantly, major Chinese electric vehicle makers intend to use sodium-ion batteries in new vehicle models this year.
Sodium-based chemistries
Sodium-ion batteries are (today) yet again lower in energy density than LFP batteries. Commercialized LFP batteries from CATL have an energy density of 160 Watts per kilogram. One of the first commercial sodium-ion batteries, from Chinese manufacturer HiNa, will have an energy density of 145Wh/kg, well below the 255Wh/kg of CATL’s next generation of NMC batteries. That density advantage may not endure: sodium-ion batteries have been under development for years and have a lab-tested track record of improvement. New small-batch production cells from Faradion already have higher density than commercial LFP batteries, and Faradion’s prototype cells are approaching 200 watt-hours per kilogram energy density.
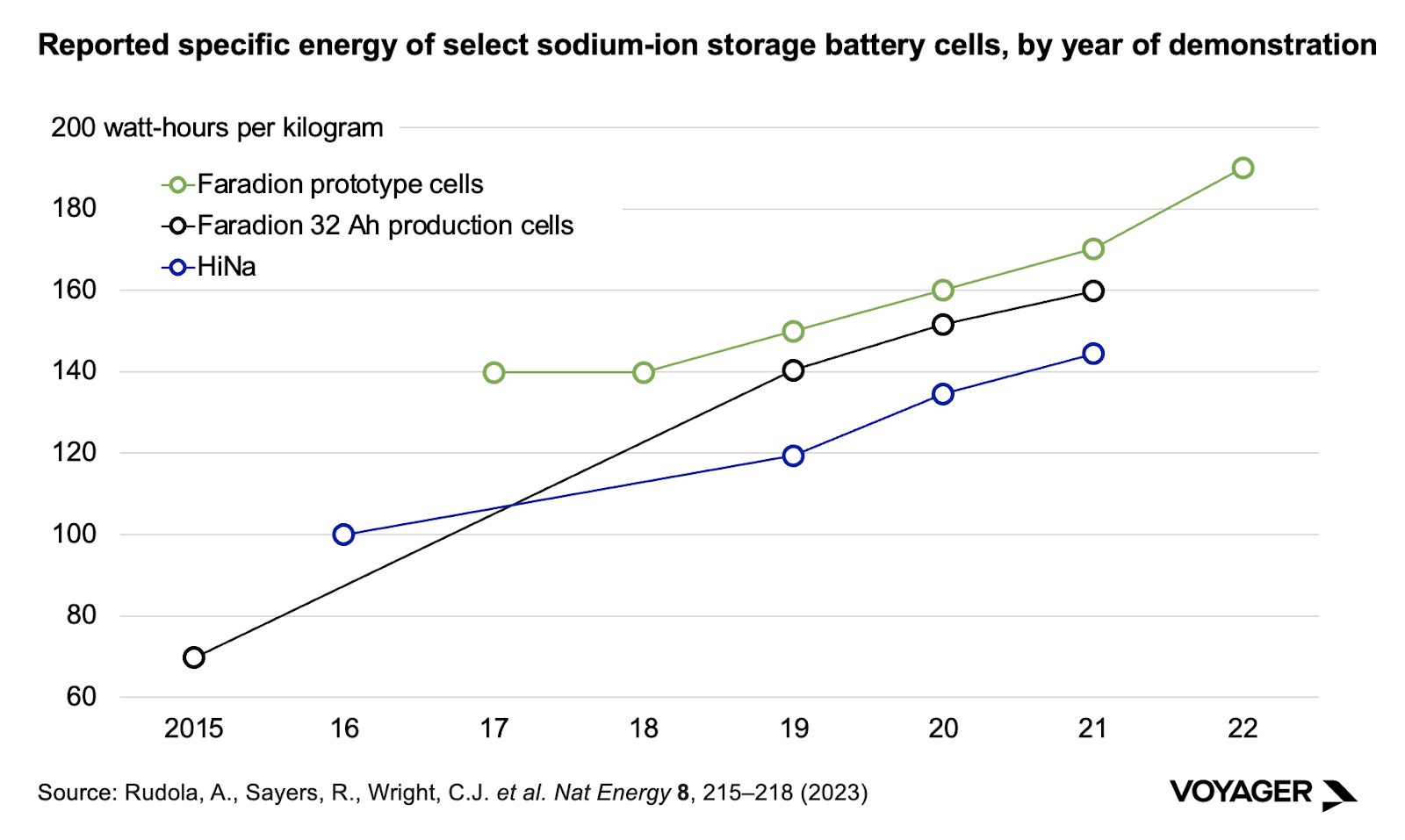
Abundance and safety are also key advantages. The sodium-ion battery supply chain relies on extremely abundant metals, meaning that manufacturers should be able to avoid shortages and chokepoints for key inputs. Sodium-ion batteries also have major safety advantages over NMC batteries. Sodium-ion batteries can be safely discharged to zero volts, which means they can be transported without any risk of thermal runaway. And finally, there is price. Sodium-ion batteries could price as much as 50% below comparable LFP batteries.
These battery developments are incredibly exciting. Low-cost, high-safety sodium-based batteries are well-suited for stationary storage applications, where their safety features make them suited for building-integrated uses and their energy density tradeoff is least impactful. Lithium iron phosphate batteries will be in vehicles, of course, but also in grid applications, where their advantages are similar to - but weaker than - sodium-ion. Low-cost, zero-transport-risk sodium-based batteries will make their way from vehicles into other applications. Lithium iron phosphate batteries will be in vehicles of course, but also in grid applications. Sodium-ion batteries will likewise be used in stationary storage applications, with their safety features making them suited for building-integrated uses.
This proliferation of chemistries is also positive for NMC batteries. As LFP and sodium-ion batteries improve, innovation in NMC batteries can focus that chemistry on higher-value use cases such as high-performance road transport – and eventually, air transport too.
The gains from battery chemistry will not be limited to battery manufacturers. Lower costs and greater safety create better products, and those better products create a bigger ultimate market for energy storage. Energy storage project developers and asset owners will build that market. Battery deployment at exponential scale in vehicles and attached to the grid will create the need for an ecosystem of specialized software and analytics firms. Investors will create dedicated financial funds and investment structures tailored to emerging energy storage markets. Reuse and recycling businesses will thrive on an abundant supply of batteries entering the circular economy. Energy storage is already a market, but as it expands it will create new markets for optimizing, financing, reusing, and recycling batteries at scale.
Learning and unlearning from solar
Voyager has collective decades of experience with solar power technology and markets. While we are wary of over-reliance on pattern-matching, we value solar’s example as another fast-growing, technology-driven, manufacturing-intensive energy technology on its way to ubiquity.
Solar entered its own commodity price spike period as it achieved global scale, in the later years of the 2000s. At that time, refined silicon pure enough to be used to make solar panels was scarce and spot prices were exceptionally high – in much the same fashion that lithium was scarce and expensive a year ago. The result, for solar, was a meaningful substitution of alternative solar semiconductors that used little or no silicon. By 2009, amorphous silicon, copper indium gallium selenide, and cadmium telluride solar modules were 17% of all photovoltaic production by wattage.
But then, solar-grade polysilicon supply expanded greatly, and prices dropped precipitously, to the point that alternative solar semiconductors lost market share steadily for a decade. Only cadmium telluride, manufactured almost exclusively by First Solar, has expanded market share again after losing it – but remains less than 5% of global module production, down from 13% in 2009.

Thin-film semiconductors do hold some operational advantages. One is a lower temperature coefficient than crystalline silicon – that is, they lose less efficiency for every degree of increase in temperature than silicon does. The second is better performance in lower-light conditions, such as early and late in the day, under dust or cloud cover, or at higher and lower latitudes. In aggregate these advantages can be non-trivial and add a percentage point or two to solar energy yield per year. Emerging paradigms can be lightweight and far more flexible than mass-market polysilicon solar panels.
However, operational advantages only matter if they result in either a lower cost of delivered power for the same rated output or result in higher internal rates of return for asset owners. In that sense, crystalline silicon solar has brute-forced its way into dominance: it is cheap, it is ubiquitous, and it prices out. There are cases where other tech can out-compete it, but in the main, it wins on cost, and on price.
There are two lessons here. The first, and rather basic one, is that market assumptions of decades-long scarcity in material inputs have a way of upending themselves. The cure for high prices is high prices, after all, and the market response to scarce and expensive solar silicon resulted in it becoming abundant and cheap. To meet the need for critical battery metals, while reducing emissions from the production of those materials, Voyager has already invested in Aepnus, which makes novel electrolyzers that improve the recovery of critical minerals in battery recycling, and Atlas Materials, which has developed a process for low-carbon to carbon-negative nickel refining. Low-volume alternatives that exist on no other justification than price will likely struggle to compete when a high-volume incumbent finds itself in input abundance.
The second is that low cost is almost a technology factor in and of itself. There may be competing technology options for a specific purpose, each optimized in a different way or to a different degree, but low cost and high availability are often the most important factors in determining market share. Couple low hardware cost with low financing and insuring costs, as incumbent tech becomes less and less of a perceived risk, and a virtuous cycle begins. This was the condition that crystalline silicon solar created for itself almost 15 years ago, and which remains the case.
While some of solar’s lessons apply to batteries, some do not. Solar’s low cost, high availability, high reliability, and the acceptance of lenders and underwriters are all relevant for batteries. But where the differences in solar semiconductor performance are often decided on the financial margin, Voyager believes that battery distinctions along factors including weight and performance are significant enough for multiple markets to emerge.
A battery providing enough power to sustain flight, and a battery that provides frequency regulation and voltage support, and a battery that serves residential buildings in locales with extreme annual temperature variations, all store electrons. But they do different things for the market, and have different priorities: several factors’ difference in specific energy density, near-instantaneous power discharge, or very low risk of thermal runaway. That gives each technology not just a market to capture, but a market to create by providing a differentiated service. It also means that with sufficiently differentiated services, acceptable tradeoffs can be made – such as accepting large physical footprints for lower-efficiency stationary batteries.
The shape of things to come
Battery industry scale, and battery industry innovation, will remake our relationship to energy and re-imagine the world’s power system, its transport networks, and our universe of electronic devices. Advances at the high-volume end of storage batteries provide the material foundation for ubiquity; low cost, high safety, and reliability create the market conditions for storage to enter every energy grid and transport system. At the macro level, batteries’ ubiquity will entirely reshape familiar markets – energy and transportation obvious among them. At the object level, emerging form factors for energy storage promise product experiences beyond what we can imagine today.
As we mentioned in Voyager’s 2022 letter Electrons for Tomorrow, electrification of light-duty transportation will upend established markets and applications for electricity. With automotive storage batteries dominating total energy storage deployments, vehicles will soon be more than just a demand sink for stored electrons. By some estimates, electric vehicle batteries will be able to satisfy short-term demand for grid-scale energy storage as soon as 2030. Mobile batteries can create a mobile electricity grid, entirely reshaping power markets and enabling individuals, fleets and businesses to participate in a dynamic and transactive market for electricity. Fleets of electric vehicles will have the ability not just to back up the grid during times of peak power demand, but also to enrich their owners and operators for dispatching power from vehicle-to-grid. Voyager portfolio company Powerline matches the real-time markets for electricity and mobility, enabling electric vehicles to optimize the application of their stored energy: drawing power, delivering power, or storing power for longer durations. In this scenario, electric vehicles become power plants on wheels.
Voyager expects one other development that may, quite literally, enable batteries to break out of their rigid form-factors and expand into a much wider range of applications: flexible batteries. Currently, all batteries are constrained into bulky and stiff shapes, cylindrical cells or rectangular pouch cells. Flexible batteries would rewrite 150 years of assumed possibilities for energy storage. Flexible batteries could be integrated seamlessly into our clothes, devices, and any unused space where power is needed: storing energy everywhere to enable us to electrify everything. Voyager is an investor in Anthro Energy, which is commercializing a novel structural battery that is twistable, safe, and energy-dense - precursors to ubiquitous energy storage.
With the arrival of physically flexible energy storage, batteries will become more than universal: they will become integrated into our lives. At global scale, batteries will enable us to decarbonize the grid and transportation and allow us to carry our own energy with us reliably and seamlessly. Energy storage will build onto our grids and bolt onto our cars, into our homes and pockets, and onto our wrists. Batteries are a foundation for our decarbonized future.